BACTERIOPHAGE: AN OLD FRIEND RETURNS TO BATTLE SUPERBUGS IN THE POST-ANTIBIOTIC ERA
By: Rizki Auliah Bakri, Dhiny Reskita Ayu, Muhammad Fiqhi Hardiansyah, Adhella Menur
The growing threat of antimicrobial resistance (AMR)
Antimicrobial resistance (AMR) has been acknowl-edged to be one of the top three major public health threats by the WHO. AMR is potentially the greatest public health threat of our time, surpas-sing COVID-19 due to its ongoing and escalating impact. AMR affects not just human health but also animals, crops, and the environment. In 2019 alone, it was estimated that 1.27 million deaths worldwide were directly linked to AMR. The O’Neill Review on AMR estimates that 10 million people will die from AMR each year by 2050. The review also estimates that the economic impact of AMR could reach $100 trillion or reduce the global gross domestic product by 3.8% by 2050.
Antibiotics, a specific class of antimicrobials, are primarily used to treat bacterial infections and are far more commonly used than other antimicrobial classes. Antibiotic resistance, however, is a natural phenomenon that predates human use of antibiotics. In their shared ecological niche, bacteria and antimicrobials have evolved side by side, with bacteria developing mechanisms to defend against the effects of antibiotic molecules. Antibiotics typically target one of four critical components of a bacterial cell: the cell wall, cell membrane, protein synthesis, or nucleic acid synthesis. To evade these effects, bacteria employ various strategies.
The rapid global spread of “superbugs”—microorganisms resistant to most known antimicrobials—has elevated the threat of drug-resistant pathogens to a critical and alarming level. The term “ESKAPE” is an acronym representing six highly drug-resistant bacteria: Enterococcus faecium, Staphylococcus aureus, Klebsiella pneumoniae, Acinetobacter baumannii, Pseudomonas aerugino-sa, and Enterobacter. These pathogens include some of the most dangerous superbugs encoun-tered today, such as vancomycin-resistant Entero-coccus (VRE), methicillin-resistant S. aureus (MRSA), carbapenem-resistant K. pneumoniae (CRKP), multidrug-resistant (MDR) Acinetobacter, MDR P. aeruginosa, carbapenem-resistant Entero-bacterales (CRE), and ESBL-producing Enterobac-terales. Moreover, MDR tuberculosis (MDR-TB) and extensively DR-TB (XDR-TB) further amplify the challenges facing public health efforts worldwide.
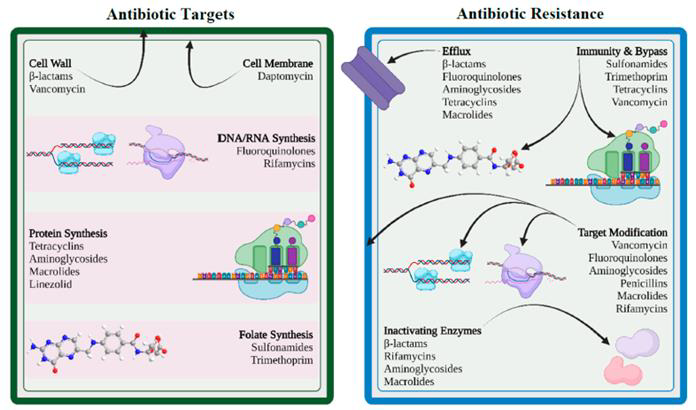
Over the decades, the surge of antibiotic resistance has been largely fueled by human activities. These include the misuse and overuse of antibiotics in medicine, the food industry, and animal husbandry. The dwindling antibiotic pipeline further exacerbates this issue as pharmaceutical companies increasingly opt out of antibiotic research and development.
The dry pipeline of novel antibiotic development
Ninety-six years ago, Alexander Fleming returned from holiday to find Penicillium growing on Petri dishes in his basement laboratory at St. Mary’s Hospital in London. This serendipitous discovery marked the beginning of the “golden age” of anti-biotics, which started in the 1940s and lasted over four decades. Over 40 antibiotics were discovered and introduced into clinical practice during this time. Resistance to individual antibiotics was not a major concern, as newer drugs with enhanced pharmacokinetic and pharmacodynamic properties were rapidly developed, fuelling a cycle of discovery, use (and overuse), and the inevitable emer-gence of resistance. By the 1990s, however, the damaging effects of this cycle had become appar-ent. The introduction of truly novel antibiotics had slowed significantly, giving rise to what is often described as a “dry pipeline” in antibiotic research and development. Most antibiotics introduced in recent years are either modified versions or combinations of existing compounds, highlighting the increasing difficulty of discovering entirely new classes of antimicrobial agents.
So, why is it so challenging to develop new antibiotics despite technological advancements? Sadly, the obstacle is not just scientific but also regulatory and economic. Novel drugs must go through the challenging process of proving their efficacy, safe-ty, favorable pharmacokinetic profile, and cost-effectiveness. This process is lengthy, expensive, and often fails, making pharmaceutical companies hesitant to invest in antibiotic research. Antibiotics are typically prescribed for short treatment durations, must be regulated under strict stewardship programs, and are constantly threatened by the emergence of resistance— all factors that reduce their profitability. Additionally, antibiotics must be used sparingly to limit the development of resistance and cannot be sold in large quantities. Compared to other, more expensive treatments, they are also relatively low in price. This combination of low sales volumes and low prices has significantly limited profit potential, leading many pharmaceutical companies, including Novartis, Sanofi, AstraZeneca, and GSK, to exit antibiotic research. However, a 2022 WHO report provided some optimism, noting 80 new antimicrobial drugs in the clinical pipeline, including 46 traditional and 34 non-traditional agents. While this offers hope, it will take time for these drugs to reach patients in need, with risks of failure, limited advantages over existing antibiotics, and the persistent challenge of drug resistance. Therefore, there is an urgent need for alternative therapeutic strategies to battle re-sistant bacteria. One promising option is revisiting an old friend: bacteriophages, or phages.
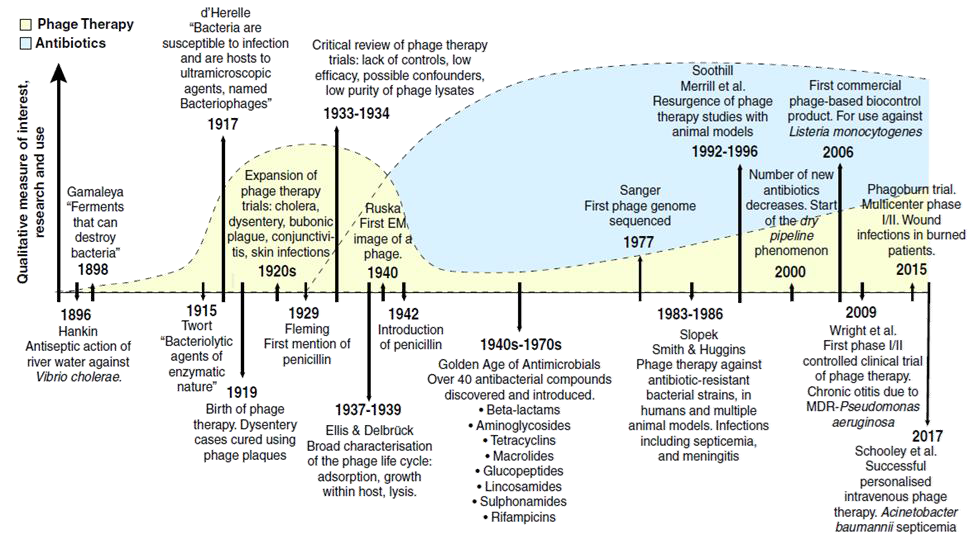
Bacteriophages: An alternative weapon against drug-resistant bacteria
In early 1896, British bacteriologist Ernest Hankin observed antibacterial activity in the Ganges and Jumna rivers in India. He found that an unknown substance seemed to possess antibacterial properties, reducing the spread of cholera in nearby villages. The “official” discovery, however, is partly credited to British pathologist Frederick Twort, who, in 1915, described a similar phenomenon while studying Staphylococcus bacteria. He published his findings in a letter to The Lancet but mistakenly identified the agent as a “dissolving sub-stance” secreted by bacteria. Two years later, French microbiologist Felix d’Herelle published a paper that accurately described the viral nature of this invisible anti-Shiga microbe. He isolated it from the feces of recovering dysentery patients and named it a “bacteriophage”—from the Greek “bacteria” and “phagein” (to eat)—meaning “bacteria eater.”
Bacteriophages, or phages, are defined as viruses that exclusively target, infect and replicate within bacterial cells, leading to cell death and lysis. They are the most abundant and widespread organisms on Earth, with estimates suggesting over 1031. Like other viruses, phages are obligate intracellular parasites that depend on the bacterial host’s machinery for reproduction. According to the International Committee on the Taxonomy of Viruses, phages are classified into one major order, 19 families and 31 genera. Notably, the majority (96%) of identified phages are tailed dsDNA phages belonging to the families Myoviridae, Podoviridae, or Siphoviridae, which resemble miniature lunar landers.
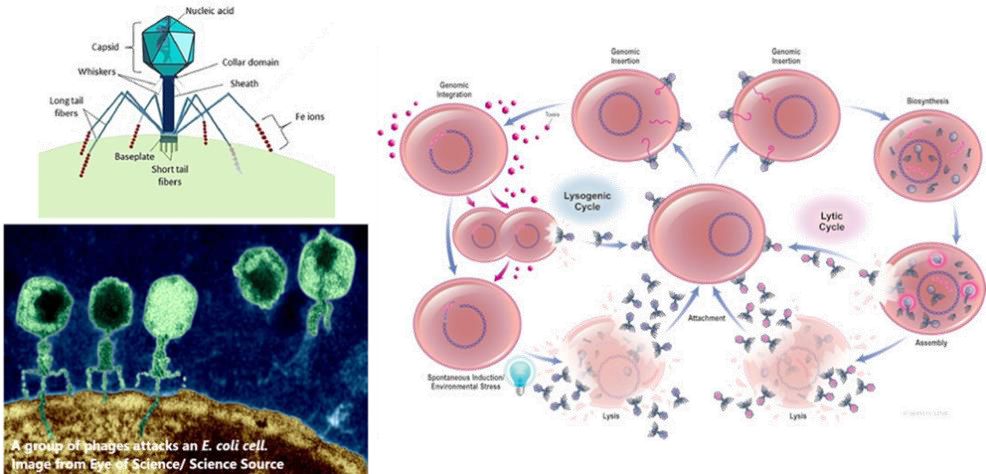
Phages have complex relationships with their bac-terial hosts. They can be classified as virulent or temperate based on their life cycles. Virulent phages follow the lytic cycle, destroying the bacterial cell directly, while temperate phages follow the lysogenic cycle, integrating their genetic material into the bacterial DNA without immediately killing the host. Temperate phages may carry resistance or toxin genes, protect their host from additional phage attacks, and modify bacterial traits. For therapeutic applications, virulent phages—those undergoing exclusively lytic cycles—are the primary and most suitable choice. However, researchers have proposed that genetically engineered temperate phages could serve as gene delivery vehicles, enabling the development of programmable, gene-specific antimicrobials. Further studies are needed to ensure that temperate phages can be considered an option for phage therapy. Since the promising initial reports in 1919-1940, enthusiasm for phage therapy declined with the advent of anti-biotics, though treatment centers have persisted in Eastern Europe and the former Soviet Union. How-ever, it is now regaining interest in the post-antibiotic era.
While antibiotics generally exert broad-spectrum effects, phages are highly specific, attacking only their designated bacterial hosts. This precision, combined with their ability to co-evolve with bacterial populations, positions phages as a promising tool in combating antibiotic-resistant infections. A phage initiates its attack by using tail fibers to at-tach to specific receptors on the bacterial cell wall. It then injects its genetic material into the host, hijacking the bacterial cellular machinery to pro-duce new phages. As replication proceeds, the bacterium becomes filled with newly formed phages until it eventually bursts (lysis), releasing 50–200 new phages. These newly released phages infect nearby bacteria, continuing the cycle. Once the bacterial host is eradicated, phages naturally cease functioning, making them a self-limiting therapeu-tic option. Since phages are primarily composed of nucleic acids and proteins, they are inherently non-toxic. However, their interactions with the immune system warrant careful evaluation. Additionally, bacterial debris and endotoxins released during lysis must be managed to prevent potential ad-verse events.
To address the limitations of narrow-host-range phages, phage cocktails have been developed. These cocktails combine multiple phages with different receptors and characteristics, allowing them to complement each other in targeting the same bacterial species and strains. By employing diverse phages, these cocktails enhance efficacy and help slow the emergence of phage-resistant bacteria. Uniquely, although phage resistance may occur, it often has a beneficial effect, as bacteria, in their effort to defend against phages, can become re-sensitized to antibiotics they were previously resistant to.
Applications and research on phage therapy for MDR bacteria
Phage therapy has been employed under compassionate use through emergency investigational new drug applications or temporary use authorizations. It is typically considered a last-resort treatment for patients with prolonged infections who have not responded to multiple courses of antibiotics. Several centers worldwide are dedicated to phage therapy research and applications. These include the Eliava Institute (Tbilisi, Georgia), Queen Astrid Military Hospital (Brussels, Belgium), Centre Hospitalier Universitaire de Lyon (Lyon, France), Westmead Institute for Medical Research (Sydney, Australia), HUJI-HMC Phage Therapy Institute (Jerusalem, Israel), and the Center for Innovative Phage Applications and Therapeutics (IPATH) in San Diego, US. Other institutions in the US, such as Baylor College of Medicine, Mayo Clinic, and Johns Hopkins University, have also established clinical phage therapy centers.
The typical process of phage therapy begins when a clinician takes a bacterial sample from the patient, isolates the pathogenic strain, and sends it to a specialized institution or laboratory to request phages. The laboratory conducts a phagogram to test the activity of different phages against the bacterial strain. If one or more phages prove effec-tive, they are produced, purified, and sent to the clinician for administration. If no active phages are found in the existing collections, they may initiate phage hunting, exploring environments such as wastewater, sewers, or treatment plants to identify new phages. These are then produced and provid-ed for treatment. Globally in 2021, at least 137 phage targets were identified across 135 academic and commercial phage facilities, along with 92 or-ganizations, including phage banks and biotech-nology companies. Due to this individualized pro-cess, there is often a delay between the request and patient administration. The encouraging news is that several institutions have reported promising outcomes, demonstrating both the safety and effi-cacy of phage therapy in local and systemic admin-istration based on their experiences.
Table 1. Advantages, disadvantages, and similarities of phage therapy compared to antibiotic therapy (source: Gor-dillo Altamirano FL, Barr JJ. 2019. https://doi.org/10.1128/CMR.00066-18).
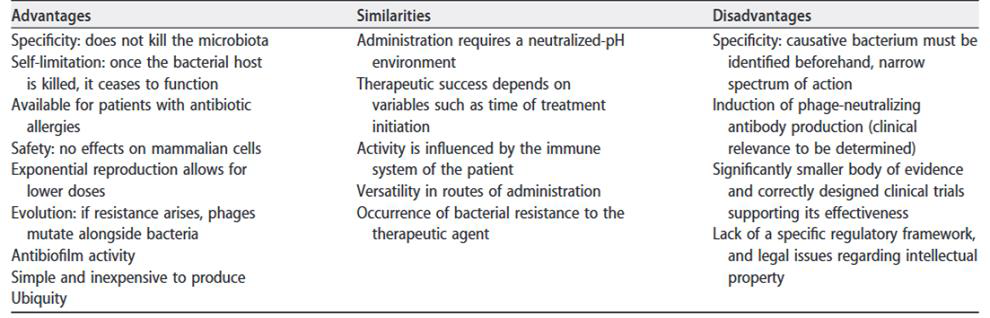
Adding to the good news about phage therapy, in 2016, Paul E. Turner, a Professor of Ecology and Evolutionary Biology at Yale University, and his team isolated a lytic phage called OMKO1 from a Connecticut pond. OMKO1 targets MDR P. aeru-ginosa by attaching to its cell membrane at the site of an efflux pump mechanism, which the bacteria evolved to expel antibiotics. Experimental evolution revealed a trade-off: when P. aeruginosa devel-oped resistance to OMKO1, it altered its mem-brane, reducing the pump’s efficiency. This change rendered the bacteria more susceptible to several classes of antibiotics. Two years later, OMKO1 was used to treat a 76-year-old patient with a MDR P. aeruginosa mediastinal and aortic graft infection. Combined with the antibiotic ceftazidime, a single phage application successfully resolved the infection without recurrence, highlighting the therapeutic potential of phage-antibiotic combinations.
Despite the promising results, it is important to recognize that available case studies are often biased toward positive outcomes. Small sample sizes, inconsistent study designs, and the absence of standardized treatment protocols limit the current evidence. To establish phage therapy as a widely accepted treatment option, larger-scale clinical trials are essential to confirm its safety, efficacy, and applicability to various infections. Additionally, these trials should focus on developing standard-ized guidelines for phage selection, dosing, and administration, which are currently lacking in the field. From 2015 to 2017, the Phagoburn study evaluated a 12-phage cocktail for treating burn wound infections caused by P. aeruginosa in 25 patients. It was the first phage therapy trial con-ducted under GMP and GCP standards. The study reported no adverse effects of the phage cocktail and demonstrated a significant reduction in patho-gen load within the wounds. However, this reduc-tion occurred at a slower rate than in the control group, which received standard care with 1% silver sulfadiazine cream. The study’s limitations included small sample size, reduced phage titers after man-ufacturing – leading to lower-than-intended doses being administered, and bacterial resistance to low phage doses. The authors highlighted the need for further research to address these issues. As of No-vember 2024, ClinicalTrials.gov lists 52 clinical trials investigating phage therapy. These include 28 Phase I or Phase II trials, 16 combined Phase I/II trials, and eight Phase III trials.
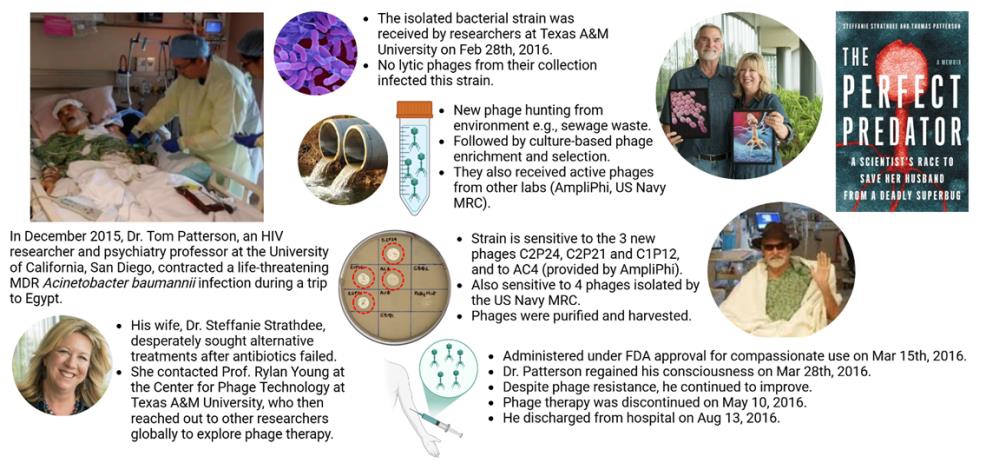
The future of bacteriophage therapy in the post -antibiotic era
It’s a sentimental story of an old friend from the pre-antibiotic era, shedding light on our battle against superbugs in the post-antibiotic era. The success of phage therapy relies on carefully select-ing specific bacteriophages, making rapid and ac-curate pathogen detection and extensive phage libraries essential. Co-administration of antibiotics as adjuvants is recommended to prevent infections by non-target bacteria and to aid in eliminating bacteria already weakened by phages. With a greater understanding of phage biology, advancements in technology, and higher medical standards, the future of phage therapy looks bright.
References
- Aranaga, C., et al., 2022. Phage therapy in the era of mul-tidrug resistance in bacteria: a systematic re-view. International journal of molecular sciences.
- Brives, C. and Pourraz, J., 2020. Phage therapy as a potential solution in the fight against AMR: obstacles and possible futures. Palgrave Communications.
- Chan, B.K., Turner, P.E., et al., 2018. Phage treatment of an aortic graft infected with Pseudomonas aeruginosa. Evolution, medicine, and public health.
- Chung, K.M., Nang, S.C. and Tang, S.S., 2023. The safety of bacteriophages in treatment of diseases caused by multidrug-resistant bacteria. Pharmaceuticals.
- Dutescu, I.A. and Hillier, S.A., 2021. Encouraging the development of new antibiotics: are financial incentives the right way forward? A systematic review and case study. Infection and drug resistance.
- Gordillo Altamirano, F.L. and Barr, J.J., 2019. Phage therapy in the post-antibiotic era. Clinical microbiology reviews.
- Ho, C.S., et al., 2024. Antimicrobial resistance: a concise update. The Lancet Microbe.
- Olawade, D.B., et al., 2024. Phage Therapy: A targeted approach to overcoming antibiotic resistance. Microbial Pathogenesis.
- Salam, M.A., et al., 2023. Antimicrobial resistance: a growing serious threat for global public health. Healthcare.
- Strathdee, S.A., et al., 2023. Phage therapy: From biological mechanisms to future directions. Cell.
- https://asm.org/articles/2022/august/phage-therapy-past,-present-and-future
- https://wellcome.org/news/why-is-it-so-hard-develop-new-antibiotics
Most Commented